To what extent are wastewater treatment systems a gateway for microplastic particles in the aquatic and terrestrial environments?
Abstract
Sewage treatment plants are designed to handle wastes that include several types of pollutants, including microplastics (MPs), which are synthetic polymer materials fragmented to sizes < 5 mm. Investigating the abundance and removal efficiency rates of MPs in wastewater treatment plants is important because the water body to which plant effluents are discharged may be adversely affected by the released MPs. Therefore, the abundance, characteristics, and removal of MPs at two sewage treatment plants located on the southwest coast of Norway are studied. Twenty-four samples were collected across two sampling sessions by using an ad hoc-designed plastic-free water sampling device, which was improved by applying a cascade of certified stainless-steel sieves. A combined sequence of enzymatic and strong oxidative incubations was performed for sample preparation. The obtained samples were chemically characterized and quantified using thermoanalytical techniques. The overall amounts of polymers in the inlet wastewater and outlet water were 366-616 μg/L and
Keywords
INTRODUCTION
The introduction of plastic materials into our everyday life has indisputably increased our quality of life. Owing to their intrinsic flexibility, plastic materials can be tailored to cater to a wide range of demands, resulting in increased production and usage of plastics in many applications worldwide. However, the massive release of plastic litter and its slow degradation rate are affecting the environment. Microplastics (MPs) are defined as plastics whose sizes are smaller than 5 mm (US NOAA)[1]. They may be either primary or secondary in origin. Primary MPs are manufactured intentionally and used in various applications, including cosmetics and industrial applications such as sandblasting and surface maintenance. Secondary MPs are generated by the photochemical and physical fragmentation of larger macroplastics[2]. While our understanding of the fate and effects of MPs in the marine environment is advancing, knowledge gaps pertaining to plastics in freshwater and terrestrial environments persist.
Sewage treatment plants (STPs) collect sewage from households, industries, and artisanal activities. MPs in sewage may originate from diverse activities, including washing of clothes, floor cleaning, mechanical street washing, plastic beads used in personal care products, and debris from other plastic objects accidentally or deliberately disposed into drains[3]. STPs are complex systems that employ chemical, physical, and biological processes to release an eco-friendly final discharge into terrestrial or aquatic environments. The existing STPs are not engineered to manage plastic microlitter[4]. A significant, but variable, fraction of the MPs arriving at an STP are retained in its final discharge[5]. Therefore, the effluents discharged by STPs have emerged as an important pathway for the entry of plastic microlitter into aquatic environments and, possibly, terrestrial environments through the spreading of sewage sludge on farmland[6]. Nevertheless, substantial data to assess the relevance of STP effluent as a contributor to plastic pollution are lacking, especially in relation to the data available for other sources.
The main objective of this research is to develop a standardized method for collecting, quantifying, and characterizing MPs to accurately estimate the inlet/outlet flux. The results of this work may help clarify the fate and behaviour of MPs in complex STP systems. The focus is on sewage sludge, a valuable byproduct of the sewage treatment process that is utilized in agricultural production.
MATERIALS AND METHODS
Sampling sites
This study was performed at two STPs, namely the North Jæren STP (NJ-STP) and Grødaland STP (GR-STP), located in Rogaland County on the southwest coast of Norway. These plants employ different rinsing systems. The North Jæren STP mainly collects household wastewaters and subjects them to bar screening, grit removal, pre-aeration, primary sedimentation, chemical-physical treatment, and secondary sedimentation. Meanwhile, the Grødaland STP collects industrial discharges and subjects them to bar screening, grit removal, pre-aeration, primary sedimentation, activated sludge treatment, secondary sedimentation, and tertiary filtration. This plant has separate pretreatment systems for (a) food wastes from households and grocery chains; and (b) wastewater sludge, septic sludge, and other organic industrial wastes. Samples were obtained through the filtration of influent and effluent wastewaters. An ad hoc designed stainless-steel device was engineered to filter large volumes of wastewater and separate plastic particles into two dimensional classes: > 300 µm (D1) and 300 µm < D2 < 10 µm [Supplementary Figure 1].
The sampling device consisted of two removable plain Dutch weave stainless-steel sieves having a diameter of 30 cm and mesh sizes of 300 and 10 μm, separately. The mesh screens were fixed at the two ends of a 40-cm-long stainless-steel pipe to obtain a filter cascade with the largest mesh size at the top being the first filter unit in touch with the water sample. This design facilitated both the collection of a relatively large volume of wastewater and in situ fractionation of MPs. A flow meter was installed at the end of the device to precisely measure the volume of the sampled wastewater. The efficiency of the designed sampling device was validated using replicates of a 100-L tap water sample spiked with 900- and 50-μm-sized polyethylene particles obtained from Goodfellow Ltd. The contributions of each of the size classes of plastics to the spiking mixture were set by mass. To determine the efficiency of the sampling device, 200 mg of the Ø =
Sampling
The inlet raw water and the outlet wastewater of the two studied STPs were sampled in March and July 2017, and three replicates/site per sampling session were obtained. To reduce any potential variability associated with rain regimes or possible changes in the main flux entering the STPs, the sampling process was repeated for two days. The obtained samples were pooled together. Different volumes of untreated and treated wastewater were sampled [Supplementary Table 1]. As the sampling process, wastewater was pumped from the water stream into the sampling device by using a submersible stainless-steel pump coupled to a rotating bronze head. Influents were collected after the wastewater was passed through a coarse screen (1 cm mesh size) to prevent larger debris from clogging or damaging the pump head. Depending on the status of the wastewater, each sampling event lasted 1-2 h with a maximum flow rate of 5 L/min. After each sampling session, both filters were removed, and their contents were quantitatively transferred to pre-cleaned glass jars by back-flushing the filters and using pre-burned stainless-steel spatulas. To prevent any possible cross-contamination, the sampler was flushed with tap water between sampling events. In total, 24 samples were collected in this study.
Furthermore, a total of 5 L of 5-day digestates were collected from both STPs by pooling together aliquots from the dryer at intervals of five days. The sewage sludges generated by both STPs were further subjected to anaerobic digestion, leading to a decrease in the proportion of organic material by approximately 50%. The anaerobic digesters of both plants received a certain amount (approximately 10% of the dry mass or DM input) of external material, such as sewage sludge, sludge from the aquaculture industry, and food waste, which may have contributed its own MP load. Generally, at the GR-STP, the digestate is dewatered and utilized in agriculture as a soil amendment. Meanwhile, at the NJ-STP, the digestate is dried at 105 °C to approximately 90% DM, additional plant nutrients are added, and the product is marketed as an organic or organic-mineral fertilizer. The anaerobic digestion and dewatering processes were not likely to affect the MP, but at 105 °C, the effects of these processes on MP shape and degradation cannot be ignored. These effects have not been investigated thus far. Solid samples were collected either as dried digestate (NJ-STP) or dewatered digestate (GR-STP). Five litres of digestate samples were collected on five different days from both STPs, and equal aliquots of all samples were pooled and used for analysis. Sample collection was performed using a stainless-steel shovel, and the collected samples were homogenized and stored in glass containers under dark and cold (-20 °C) conditions before further processing. The storage containers were rinsed with Milli-Q and muffled at 500 °C for 3 h beforehand.
Sample preparation
Wastewater
The material trapped by the filter was dried in an oven at 60 °C for 72 h and weighed thereafter. To remove natural organic and inorganic materials before the characterization and quantification analyses of the plastic fragments without damaging their polymer structure, samples were prepared by enzymatic digestion followed by iron-catalysed oxidation incubation in sequence. All the applied enzymes (Sigma Aldrich, Germany) were of technical grade and filtered (0.2 µm) before use. The sample purification process was started by adding 10 mL of sodium dodecyl sulphate (SDS) (5% w/vol) per gram dry weight (DW) of the sample. The samples were then placed in a 300-mL beaker and incubated at 50 °C for 6 h. The SDS-incubated samples were vacuum-filtered on a pre-muffled (500 °C, 3 h) 125 mL glass crucible filter (porosity 4, ROBU, Germany). The digestates were vacuumed off and the remaining material trapped on the sintered glass filtration surface was rinsed by flushing it with 50 mL of GF/A-filtered (1.2 µm) ultrapure water. Approximately 10 mL of a Viscozyme and Cellulclast mixture per gram of sample DW (1:10; v:v) in acetate buffer (0.1 M; pH 4.8) was added to the same crucible, and the resulting sample was placed in an ultrasound bath for 5 min and incubated for 36 h at 50 °C. The digestates were then vacuumed off using the same crucible filter, and the residual material was rinsed again with 50 mL of ultrapure water, digested for 48 h at 30 °C with 10 mL of protease per gram of sample DW (Sigma, Germany) in PBS (1:10 at pH 7.4), vacuumed off, and rinsed with ultrapure water. The digestates were then filtered through the crucible, and the residual material rinsed again with 50 mL of ultrapure water, incubated for 48 h at 30 °C with 50 mL of lipase in PBS (1:5 at pH 7.4), vacuumed off, and rinsed with Milli-Q. The digested material was then filtered through the crucible surface, and the residual material rinsed again with 50 mL of ultrapure water, incubated for 12 h at 40 °C with 50 mL of lignin peroxidase and laccase enzymes in tartarate buffer (1:5 at pH 3.0), vacuumed off, and rinsed with ultrapure water. Finally, the residual organic material was oxidized by performing the iron (II)-catalysed hydrogen peroxide (H2O2) reaction (Fenton reaction). H2O2 and iron (II) were added to final concentrations of 250 g/L and 2.5 g/L, respectively[7]. The pH of the mixture was adjusted to ~3 with NaOH. The crucible was kept in an ice bath to maintain the temperature between 30 and 40 °C. The oxidized material was vacuumed off, and the residual iron was washed away from the crucible’s surface by flushing it with 100 mL of 0.5 M HCl solution and 50 mL of Milli-Q thereafter.
Digestates
The digestate samples stored in glass containers were dried in an oven at 105 °C for 3 days (Thermolab, Norway). The residual water contents of the dehydrated samples were estimated to assess any possible dilution effects of the reagents and enzymes by following the procedure of Gomiero et al.[8]. Homogenized digestate sample aliquots of 50 g (n = 5) were subjected to a sequential combination of enzymes followed by strong oxidation treatment before chemical characterization of the processed samples. The samples were initially spiked with 50 μg of d4-PE, was used as an internal standard for all the target plastics because they were extracted using the same method. All the mass concentration values of the sludge analysed in this study were corrected for the recovery percentages of the internal standard, which ranged from 76% to 92%. Sample preparation started with the addition of 10 mL of SDS (5% w/vol) per gram DW of the sample. The samples were placed in a 1-L beaker and treated at 50 °C for 6 h on a Gallenkamp Incubator Shaker (BioNordika, Norway). The SDS-incubated samples were vacuum-filtered using a sintered glass filter funnel with a volume of 1 L (porosity 4, VWR, Norway). The digestates were vacuumed off, and the remaining material trapped on the sintered glass filtration surface was rinsed by flushing it with 100 mL of filtered ultrapure water. Then, 100 mL of a mixture of Viscozyme and Cellulclast per gram of sample DW (1:5, v:v) in acetate buffer (0.1 M; pH 4.8) was added to the same crucible, and the sample was sonicated for 5 min and digested for 48 h at 50 °C. The digestates were then filtered using the same crucible, and the residual material was rinsed again with 100 mL of ultrapure water, incubated for 48 h at 30 °C with 100 mL of protease per gram of sample DW (Sigma, Germany) in PBS (1:5 at pH 7.4), filtered, and rinsed with Milli-Q. The digestates were then filtered, and the residual material was rinsed again with 100 mL of Milli-Q, incubated for 48 h at 30 °C with 100 mL of lipase in PBS (1:5 at pH 7.4), filtered, and rinsed with Milli-Q. The digested material was then filtered, and the remaining material was rinsed again with 100 mL of ultrapure water, incubated for 12 h at 40 °C with 100 mL of lignin peroxidase and laccase in tartarate buffer (1:5 at pH 3.0), vacuumed off, and rinsed with 100 mL of ultrapure water. Finally, the residual organic material was oxidized by performing the Fenton reaction as described above. Approximately 100 mL of 30% peroxide and 2.5 g/L of iron (II) sulphate were added. The oxidized material was filtered, and the residual iron was washed off the crucible surface by flushing it with 100 mL of 0.5 M HCl solution, followed by
Analysis of plastics
Pyrolysis gas chromatograph mass spectrometer (GCMS) measurements were performed using a Shimadzu Optima 2010C GCMS equipped with an Rxi-5ms column (RESTEC, Bellefonte, PA) and coupled to a multi-shot pyrolizer [EGA/PY-3030D, Frontier Lab (Supplier: BioNordika, Norway)], as previously reported[7]. Pyrolysis was performed at 590 °C. Eight polymers, namely polycarbonate (PC), polypropylene (PP), polyvinyl chloride (PVC), polystyrene (PS), polymethyl methacrylate (PMMA), PE, polyethylene terephthalate (PET), and polyamide (PA)6, were selected for chemical characterization and quantification. Standards of polymers with purity > 99% were purchased from Goodfellow Ltd. (Huntingdon, England). These standards were used to set up the calibration and quantification curves. To identify and quantify the target plastics, specific indicator ions were selected, as described and identified previously [Supplementary Table 2][7]. For calibration, between 0.2 and 420 µg of the polymer standards were weighed using an XPE205 DeltaRange Mettler Toledo balance (Germany).
In the water and sludge samples, d4-PE was used as the internal standard. All mass concentration values reported herein were adjusted for recovery of the internal standard added to the samples before extraction. Internal standard recoveries ranged from 81% to 94 %. The limit of detection (LoD) was calculated as described by Hermabessiere et al.[9].
Quality control/Quality assessment
Synthetic clothing was avoided by the personnel involved in this study. The use of glass materials was prioritized during sample preparation, and all work surfaces were cleaned with ethanol filtered over a
Calculations
The removal efficiency of plastics with sizes > 10 μm was calculated using the following formula:
RESULTS
The effectiveness of the designed sampler was confirmed using 50-µg spikes of the 900- and 50-µm-sized PE particles. The calculated recoveries ranged from 83% to 99%, indicating the suitability of the designed sampling device for the present study [Supplementary Table 3].
Some of the procedural blanks and airborne control samples of plastics were found to be slightly contaminated with plastics. Such contamination consisted predominantly of PE (≈ 11 µg) and PS (≈ 9 µg)
Figure 1. (A) Relative abundance of polymers in the inlet and outlet waters of NJ-STP; (B) Relative abundance of polymers in the inlet and outlet waters of GR-STP. NJ-STP: North Jæren sewage treatment plant; GR-STP: Grødaland sewage treatment plant.
Occurrence and distribution of MPs in influent and effluent waters of NJ-STP and GR-STP
NJ-STP Concentration µg/L | |||||||||
PE | PP | PS | PVC | PA | PMMA | PC | PET | ∑ | |
Influent March-17 | 271 ± 15 | 48 ± 4 | 32 ± 6 | 96 ± 5 | 12 ± 3 | 21 ± 4 | 11 ± 4 | 125 ± 7 | 616 ± 34 |
Effluent March-17 | 24 ± 5 | < 10 | < 8 | < 15 | < 10 | < 15 | < 11 | 11 ± 3 | 35 ± 9 |
Influent July-17 | 311 ± 12 | 55 ± 3 | 36 ± 7 | 110 ± 12 | 13 ± 2 | 24 ± 4 | < 11 | 143 ± 12 | 692 ± 31 |
Effluent July-17 | 37 ± 8 | < 10 | < 8 | < 15 | < 10 | < 15 | < 11 | 17 ± 3 | 54 ± 12 |
Procedural blanks (n = 10) | < 10 | < 10 | < 8 | < 15 | < 10 | < 15 | < 11 | < 10 | |
Airborne contamination control (n = 20) | 11 | < 10 | 9 | < 15 | < 10 | < 15 | < 11 | < 10 | |
GR-STP Concentration µg/L | |||||||||
PE | PP | PS | PVC | PA | PMMA | PC | PET | ∑ | |
Influent March-17 | 147 ± 13 | 91 ± 5 | 23 ± 6 | 70 ± 7 | < 10 | < 15 | < 11 | 35 ± 4 | 366 ± 23 |
Effluent March-17 | 22 ± 5 | 21 ± 3 | < 8 | < 15 | < 10 | < 15 | < 11 | < 10 | 43 ± 8 |
Influent July-17 | 165 ± 13 | 105 ± 10 | 26 ± 5 | 75 ± 4 | < 10 | < 15 | < 11 | 41 ± 8 | 412 ± 5 |
Effluent July-17 | 21 ± 5 | 21 ± 2 | < 8 | 15 ± 3 | < 10 | < 15 | < 11 | < 10 ± 2 | 57 ± 5 |
Procedural blanks (n = 10) | < 10 | < 10 | < 8 | < 15 | < 10 | < 15 | < 11 | < 10 | |
Airborne contamination control (n = 20) | < 10 | < 10 | < 8 | < 15 | < 10 | < 15 | < 11 | < 10 |
Estimated fluxes of MPs in NJ-STP and GR-STP
NJ-STP | GR-STP | |
MPs generated, mg/m3 wastewater | 655 | 0.19 |
MP discharged to sea, mg/m3 wastewater | 45 | 0.02 |
MPs generated per p.e. (g/p.e. × year) | 62 | 0.27 |
MPs discharged to sea per p.e. (g/p.e. × year) | 4 | 0.03 |
Significantly lower levels of plastics were observed in the effluent water, and most of the targeted polymers (PP, PS, PVC, PA, PMMA, and PC) were present in quantities lower than their detection limits. The total quantities ranged from 35 µg/L in the samples obtained in the spring sampling session to 54 µg/L in the samples obtained in the summer sampling session. In both cases, PE (68%) and PET (31%) were the most abundant polymers released into aquatic bodies. Similarly, the total amounts of MPs in the GR-STP influent samples obtained in the spring and summer sampling sessions were 366 and 412 µg/L, respectively. PE (40%), followed by PP (25%), PVC (19%), and PET (10%) were the most abundant polymers in the influent water samples obtained in the two sampling sessions [Figure 1B].
Significantly lower levels of plastics were observed in the effluent water, and most of the targeted polymers such as PS, PA, PMMA, PC, and in some cases, PET, were not detected. The total quantity of plastics ranged from 43 µg/L in the sample obtained in the spring sampling session to 57 µg/L in the sample obtained in the summer sampling session. At both STPs, PE (36%-51%), followed by PP (36%-48%) and PVC (26%), were the most abundant polymers released into marine water bodies. In terms of plastic polymer removal from wastewaters, the overall efficiencies of the NJ-STP were 78% and 83%, respectively, in the spring and summer of 2017. Overall, the removal efficiency of PVC was the highest (≈ 94%), followed by those of PP
Figure 2. MPs discharged to sea, kg/year from NJ-STP and GR-STP. MPs: Microplastics; NJ-STP: North Jæren sewage treatment plant; GR-STP: Grødaland sewage treatment plant.
Given the lower population density in the GR-STP catchment area, the discharge of MPs per person from this STP is approximately three times that in case of the NJ-STP [Figure 3].
Figure 3. MPs (g/year) per equivalent person discharged to sea, kg/year by NJ-STP and GR-STP. MPs: Microplastics; NJ-STP: North Jæren sewage treatment plant; GR-STP: Grødaland sewage treatment plant.
The differential compositions of the catchment areas allowed for a further comparison between the contributions of households and the food industry to the MP loads. Assuming that the MP contribution per household was the same in the two catchment areas, the NJ-STP figures (mostly household) indicate that the contribution of the food industry was the dominant driver of MPs in the GR-STP influent. The industry contributed an organic load corresponding to approximately 105,000 p.e. (of the total of 110,000 p.e.) to the GR-STP influent. As presented in Table 2, the MP contribution of the food industry as determined from the GR-STP influent sample was negligible compared to that of the household influent sample collected from the NJ-STP. All the analysed digestate samples contained all the targeted polymers. In the digestate solids collected from the NJ-STP, the total amount of polymers ranged from 5.2 mg/g in the sample obtained in the spring sampling session to 5.5 mg/g DW in the sample obtained in the summer sampling session [Table 3]. In case of the NJ-STP, PE was the most commonly recurring polymer in the digestate samples collected in the spring sampling session, accounting for ≈ 40% of the total MPs, followed by PET (≈ 19%), PVC (≈ 16%), and PP (≈ 7%) [Figure 4A].
Figure 4. (A) Relative abundance of polymers in digestates of NJ-STP; (B) Relative abundance of polymers in the digestates of GR-STP. NJ-STP: North Jæren sewage treatment plant; GR-STP: Grødaland sewage treatment plant.
Occurrence and distribution of MPs in biosolids of NJ-STP and GR-STP
NJ-STP Concentration mg/g DW (n = 3) | |||||||||
PE | PP | PS | PVC | PA6 | PMMA | PC | PET | ∑ | |
Sludge March-17 | 2.33 ± 0.15 | 0.41 ± 0.21 | 0.27 ± 0.09 | 0.82 ± 0.22 | 0.19 ± 0.10 | 0.17 ± 0.09 | 0.08 ± 0.02 | 1.0 ± 0.1 | 5.2 ± 0.5 |
Sludge July-17 | 2.33 ± 0.21 | 0.47 ± 0.25 | 0.31 ± 0.08 | 0.94 ± 0.31 | 0.10 ± 0.10 | 0.19 ± 0.08 | 0.05 ± 0.01 | 1.2 ± 0.2 | 5.5 ± 0.5 |
Procedural blanks (n = 5) | < 0.1 | < 0.1 | < 0.05 | < 0.2 | < 0.1 | < 0.12 | < 0.05 | < 0.1 | |
Airborne contamination control (n = 5) | < 0.1 | < 0.1 | < 0.05 | < 0.2 | < 0.1 | < 0.12 | < 0.05 | < 0.1 | |
GR-STP Concentration mg/g DW (n = 3) | |||||||||
PE | PP | PS | PVC | PA6 | PMMA | PC | PET | ∑ | |
Sludge March-17 | 1.09 ± 0.05 | 0.67 ± 0.06 | 0.17 ± 0.05 | 0.52 ± 0.05 | 0.97 ± 0.1 | 0.14 ± 0.05 | 0.07 ± 0.02 | 0.9 ± 0.1 | 3.6 ± 0.2 |
Sludge July-17 | 1.23 ± 0.06 | 0.78 ± 0.09 | 0.19 ± 0.08 | 0.55 ± 0.08 | 0.86 ± 0.1 | 0.13 ± 0.07 | 0.05 ± 0.03 | 0.1 ± 0.1 | 3.0 ± 0.3 |
Procedural blanks (n = 5) | < 0.1 | < 0.1 | < 0.05 | < 0.2 | < 0.1 | < 0.12 | < 0.05 | < 0.1 | |
Airborne contamination control (n = 5) | < 0.1 | < 0.1 | < 0.05 | < 0.2 | < 0.1 | < 0.12 | < 0.05 | < 0.1 |
The relative proportions of polymers in the summer samples obtained from the two plants were approximately the same, with only non-significant variations between the samples. In the digestates collected from the GR-STP, the total quantity of polymers ranged from 3.5 mg/g in the spring samples to
The variation of plastic concentrations was rather low in terms of either individual plastics or the sum of all plastics. Therefore, the accumulation of plastics throughout the biosolid handling process can be considered comparatively homogeneous. The reproducibility and sensitivity of the proposed method were assessed by splitting one testing sample into five parallels that were analysed separately. Analytical reproducibility stood at RSD ≈ 15% across all detected plastics. Therefore, analytical variability was only a minor source of experimental uncertainty.
DISCUSSION
The results of several studies addressing the characterization and fate of MP litter in wastewater treatment systems, conducted using custom-made sampling devices and state-of-the-art analytical technologies, are available in the literature. However, in most cases, variations in filter selection, sampling frequency, and sample volume hinder comparisons of their results, underscoring the need to promote standardization or harmonization of methodologies. Previous studies have assessed smaller volumes of STP inlet and outlet waters (≈ 50 L) compared to that in the present study. Carr et al. managed to process samples of up to 1 m3 by using tubular filters with filter pore sizes of 45 and 180 µm, which were different from those used herein[13]. The selection of particle size cut-off significantly influences study outcomes, and high MP concentrations have been observed only when finer mesh sizes have been used[14,15]. In terms of analytical approaches, vibrational microscopy-oriented techniques such as micro-Fourier transform infrared analysis
In the present study, the pyrograms of the analysed water and biosolid samples highlighted the presence of all the targeted polymers such as PVC, PE, PS, PP, PC, PA6, PET, and PMMA. These polymer types have been commonly identified in the influents and effluents of STPs. In a recent review addressing the recognition, occurrence, and removal of plastic particles across 25 sewage treatment plants, Sun et al. demonstrated the occurrence of polyester (28%-89%), PE (4%-51%), and PA (3%-30%)[18]. Polyester and PA are extensively used in synthetic clothing, while PEs are used in cosmetics products, including scrubs and creams, as well as in food packaging films[19]. Polymers such as PP, PVC, and PS were observed with relative abundances spanning from 5% to 27%. These multipurpose polymers are heavily used in the food packaging, construction, house maintenance, and automotive production industries, and they are typically found in urban environments[20,21].
The total amounts of MPs and the relative abundances of polymers at the inlets of individual STPs differed significantly, with generally lower levels recorded in case of the GR-STP. This disparity in MP concentrations in the inlet waters of the two STPs likely reflects the compositions of the respective catchment areas, with the NJ-STP serving mainly private households, and the GR-STP serving a large cluster of food industry units. Probably, the former generates larger amounts of MPs from the washing of textiles, use of cosmetics, and various other household activities. Considering the overall wastewater loads, total discharges of 100 kg and 1.4 tons of MPs/year to the Eastern North Sea were estimated for the GR-STP and NJ-STP, respectively. Furthermore, the estimated levels of MPs in the effluent waters (45-50 µg/L) of both STPs could have adverse effects on marine life in the coastal area. Nanoplastic levels and MP levels exceeding 0.14 µg/L are expected to adversely affect 5% (HC5) of key species in the aquatic ecosystem, as reported by the Norwegian Scientific Committee for Food and Environment[22]. Nevertheless, the strong Norwegian Coastal Current rapidly dilutes the effluents and facilitates their long-range dispersion to larger water bodies, resulting in temporary and locally observable expected effects[23,24].
The overall plastic removal rates of the NJ-STP and GR-TP were 78%-83% and 83%-85%, respectively, with no significant differences between the two sampling sessions. Several studies have demonstrated reductions in MPs in STPs, with considerable differences in removal efficiencies. Lv et al. reported an overall removal rate of 53% for a full-scale STP located in Eastern China, which employs two parallel alternative wastewater treatment systems[25]. Akarsu et al. obtained removal efficiencies ranging from 57% to 96% for three STPs discharging effluents into Mersin Bay, Turkey[26]. Liu et al. documented an overall removal efficiency of 64% for a conventional activated-sludge STP in China[27]. Bayo et al. (2020) reported an efficient removal rate of 90% for an urban wastewater treatment plant in Cartagena, Spain, that utilizes a system comprising a primary treatment facility and two activated-sludge reactors for sewage processing[28]. Yang et al. estimated a removal efficiency of 95% for the Gaobeidian STP in the Chaoyang District of Beijing; this STP is China’s largest water recovery plant[29].
The obtained results indicate that the majority of MPs in STPs are retained in the sewage sludge in various treatment steps. Similarly, Talvitie et al. calculated that only 20% of the MPs at the wastewater inlet were released through the STP outlet, while the remaining 80% were retained in biosolids[30]. Based on total biosolid production, the authors estimated that approximately 4.6 × 108 MPs were discharged daily from the Finnish STP. Li et al. (2018) estimated that 1.56 × 1,014 MPs per year enter the environment though reuse of the biosolids generated by a Chinese STP[31]. Carr et al. and Murphy et al. found that the biosolids generated by the skimming unit exhibited the highest abundance of MPs (4,000-7,000 MPs per kg WW), which was thousands of times higher than that in the grits and biosolids. These results indicate that the skimming stage of floating solids is an effective step for removing MPs[13,32]. Furthermore, laboratory studies strongly indicate that if sufficient removal of organic particulate materials is achieved, 98%-99% of incoming MPs of all sizes can be removed from the effluent stream[33,34]. The results of these investigations have certainly increased our awareness of the MP problem at STPs. While national guidelines for the removal of MPs from wastewater are yet to be implemented, the expected EU Urban Wastewater Treatment Directive[35] will likely result in upgraded standards for wastewater treatment at both plants, possibly requiring the removal of MPs and other micropollutants. Additionally, the STPs have been informed about the existing efficiency of MP removal, as well as available technologies for monitoring in the future. In this manner, this study has undoubtedly contributed to improving our understanding of the characteristics of plastic particles in STP inlet waters and suggested potential mitigating steps that can be implemented. Moreover, the data obtained in this study have served as the starting point for an extensive survey of the effects of MPs in wastewater sludges and soils published by the Norwegian Water Association[36], thereby expanding the national knowledge base on MPs. Furthermore, these data have facilitated a preliminary correction of exaggerated estimates of MP transfer to soil, reducing the estimated amounts of MPs entering European soils from 60-400,000 tons per year[37] to approximately 2,500 tons per year[38]. Overall conclusions based on investigations on the effects of MPs on plant growth and soil fauna[36] suggest that if sludge-based digestates are applied to soil in the amounts permitted by present legislation[39], the MPs concentrations observed in the present study will not have observable effects on soil fauna and plant growth in the short run, but long-term effects due to accumulation cannot be excluded. As a first step towards mapping the sources and routes of MPs entering and leaving STPs, this investigation provides interesting and useful data. Further efforts should focus on expanding the size range of collectable MPs in wastewater and sludge particles towards finer fractions (< 10 µm). MPs in the near-micron and nanometre range are expected to enter the body organs of living organisms more easily in aquatic systems and, subsequently, those of humans, eliciting diverse toxicological effects[40]. However, further investigations are needed to present a more complete map. The pursuit of such knowledge is advocated because it could aid the formulation of plastic pollution control and prevention measures.
DECLARATIONS
Acknowledgements
The authors are grateful to the staff of the Wastewater Treatment Plant utilities at IVAR IKS for organisational and technical assistance during the sampling campaign.
Authors’ contributions
Project administration, conceptualization, methodology, investigation, formal analysis, visualisation, writing - original draft, and writing - review and editing: Gomiero A
Investigation, formal analysis, and visualisation: Øysæd KB
Review and editing: Jaén-Gil A, Navrestad V
Conceptualization, resources, methodology, data curation, writing - original draft, and writing - review and editing: Skogerbø G
Availability of data and materials
The data that support the findings of this study are available on request from the corresponding author Gomiero A.
Financial support and sponsorship
This work was supported by the Norwegian Regional Fund for Rogaland and Hordaland (RFF-Vest) grant #260053.
Conflicts of interest
The author Skogerbø G is employed by IVAR IKS. Other authors declared that there are no conflicts of interest.
Ethical approval and consent to participate
Not applicable.
Consent for publication
Not applicable.
Copyright
© The Author(s) 2024.
Supplementary Materials
REFERENCES
1. Sheavly SB, Register KM. Marine debris & plastics: environmental concerns, sources, impacts and solutions. J Polym Environ 2007;15:301-5.
2. Duis K, Coors A. Microplastics in the aquatic and terrestrial environment: sources (with a specific focus on personal care products), fate and effects. Environ Sci Eur 2016;28:2.
3. Tian W, Song P, Zhang H, et al. Microplastic materials in the environment: problem and strategical solutions. Prog Mater Sci 2023;132:101035.
4. Wezel A, Caris I, Kools SA. Release of primary microplastics from consumer products to wastewater in the Netherlands. Environ Toxicol Chem 2016;35:1627-31.
5. Mintenig SM, Int-Veen I, Löder MGJ, Primpke S, Gerdts G. Identification of microplastic in effluents of waste water treatment plants using focal plane array-based micro-Fourier-transform infrared imaging. Water Res 2017;108:365-72.
6. Eerkes-Medrano D, Thompson RC, Aldridge DC. Microplastics in freshwater systems: a review of the emerging threats, identification of knowledge gaps and prioritisation of research needs. Water Res 2015;75:63-82.
7. Simon M, van Alst N, Vollertsen J. Quantification of microplastic mass and removal rates at wastewater treatment plants applying Focal Plane Array (FPA)-based Fourier Transform Infrared (FT-IR) imaging. Water Res 2018;142:1-9.
8. Gomiero A, Øysæd KB, Agustsson T, van Hoytema N, van Thiel T, Grati F. First record of characterization, concentration and distribution of microplastics in coastal sediments of an urban fjord in south west Norway using a thermal degradation method. Chemosphere 2019;227:705-14.
9. Hermabessiere L, Himber C, Boricaud B, et al. Optimization, performance, and application of a pyrolysis-GC/MS method for the identification of microplastics. Anal Bioanal Chem 2018;410:6663-76.
10. Magni S, Binelli A, Pittura L, et al. The fate of microplastics in an Italian Wastewater Treatment Plant. Sci Total Environ 2019;652:602-10.
11. Liu K, Wang X, Wei N, Song Z, Li D. Accurate quantification and transport estimation of suspended atmospheric microplastics in megacities: implications for human health. Environ Int 2019;132:105127.
12. Gomiero A, Strafella P, Øysæd KB, Fabi G. First occurrence and composition assessment of microplastics in native mussels collected from coastal and offshore areas of the northern and central Adriatic Sea. Environ Sci Pollut Res Int 2019;26:24407-16.
13. Carr SA, Liu J, Tesoro AG. Transport and fate of microplastic particles in wastewater treatment plants. Water Res 2016;91:174-82.
14. Leslie HA, Brandsma SH, van Velzen MJ, Vethaak AD. Microplastics en route: field measurements in the Dutch river delta and Amsterdam canals, wastewater treatment plants, North Sea sediments and biota. Environ Int 2017;101:133-42.
15. Fojt J, David J, Přikryl R, Řezáčová V, Kučerík J. A critical review of the overlooked challenge of determining micro-bioplastics in soil. Sci Total Environ 2020;745:140975.
16. Ivleva NP. Chemical analysis of microplastics and nanoplastics: challenges, advanced methods, and perspectives. Chem Rev 2021;121:11886-936.
17. Picó Y, Barceló D. Pyrolysis gas chromatography-mass spectrometry in environmental analysis: focus on organic matter and microplastics. TrAC Trend Anal Chem 2020;130:115964.
18. Sun J, Dai X, Wang Q, van Loosdrecht MCM, Ni BJ. Microplastics in wastewater treatment plants: detection, occurrence and removal. Water Res 2019;152:21-37.
19. Ziajahromi S, Neale PA, Rintoul L, Leusch FD. Wastewater treatment plants as a pathway for microplastics: development of a new approach to sample wastewater-based microplastics. Water Res 2017;112:93-9.
20. Andrady AL, Neal MA. Applications and societal benefits of plastics. Philos Trans R Soc Lond B Biol Sci 2009;364:1977-84.
21. Horodytska O, Cabanes A, Fullana A. Plastic waste management: current status and weaknesses. In: Plastics in the aquatic environment - Part I: Current status and challenges. Cham: Springer International Publishing; 2019. pp. 289-306.
22. Microplastics; occurrence, levels and implications for environment and human health related to food. Opinion of the Scientific Steering Committee of the Norwegian Scientific Committee for Food and Environment. 2019. Available from: https://vkm.no/download/18.5b7a016e16dcd89a3977dc60/1571650615867/Microplastics;%20occurrence,%20levels%20and%20implications%20for%20environment%20and%20human%20health%20related%20to%20food.pdf. [Last accessed on 25 Apr 2024].
23. Simonsen M, Lind OC, Saetra Ø, et al. Coastal transport of river-discharged radionuclides: impact of speciation and transformation processes in numerical model simulations. Sci Total Environ 2019;669:856-71.
24. Ibrekk HO, Molvær J, Faafeng B. Nutrient loading to norwegian coastal waters and its contribution to the pollution of the North Sea. Water Sci Technol 1991;24:239-49.
25. Lv X, Dong Q, Zuo Z, Liu Y, Huang X, Wu W. Microplastics in a municipal wastewater treatment plant: fate, dynamic distribution, removal efficiencies, and control strategies. J Clean Prod 2019;225:579-86.
26. Akarsu C, Kumbur H, Gökdağ K, Kıdeyş AE, Sanchez-Vidal A. Microplastics composition and load from three wastewater treatment plants discharging into Mersin Bay, north eastern Mediterranean Sea. Mar Pollut Bull 2020;150:110776.
27. Liu X, Yuan W, Di M, Li Z, Wang J. Transfer and fate of microplastics during the conventional activated sludge process in one wastewater treatment plant of China. Chem Eng J 2019;362:176-82.
28. Bayo J, Olmos S, López-Castellanos J. Microplastics in an urban wastewater treatment plant: the influence of physicochemical parameters and environmental factors. Chemosphere 2020;238:124593.
29. Yang L, Li K, Cui S, Kang Y, An L, Lei K. Removal of microplastics in municipal sewage from China’s largest water reclamation plant. Water Res 2019;155:175-81.
30. Talvitie J, Mikola A, Koistinen A, Setälä O. Solutions to microplastic pollution - removal of microplastics from wastewater effluent with advanced wastewater treatment technologies. Water Res 2017;123:401-7.
31. Li X, Chen L, Mei Q, et al. Microplastics in sewage sludge from the wastewater treatment plants in China. Water Res 2018;142:75-85.
32. Murphy F, Ewins C, Carbonnier F, Quinn B. Wastewater treatment works (WwTW) as a source of microplastics in the aquatic environment. Environ Sci Technol 2016;50:5800-8.
33. Mitrano DM, Beltzung A, Frehland S, Schmiedgruber M, Cingolani A, Schmidt F. Synthesis of metal-doped nanoplastics and their utility to investigate fate and behaviour in complex environmental systems. Nat Nanotechnol 2019;14:362-8.
34. Schmiedgruber M, Hufenus R, Mitrano DM. Mechanistic understanding of microplastic fiber fate and sampling strategies: synthesis and utility of metal doped polyester fibers. Water Res 2019;155:423-30.
35. European Commission. Urban wastewater. Available from: https://environment.ec.europa.eu/topics/water/urban-wastewater_en. [Last accessed on 25 Apr 2024].
36. A 253 Microplastics in wastewater, wastewater sludge and soil. 2020. (in Norwegian) Available from: https://va-kompetanse.no/butikk/a-253-mikroplast-i-avlopsvann-avlopsslam-og-jord/. [Last accessed on 25 Apr 2024].
37. Nizzetto L, Futter M, Langaas S. Are agricultural soils dumps for microplastics of urban origin? Environ Sci Technol 2016;50:10777-9.
38. Ljung E, Olesen KB, Andersson PG, et al. Microplastics in the ecosystem. (in Norwegian). Available from: https://www.svensktvatten.se/contentassets/7be8e202754e4011a400bcff4ed89b1c/mikSVu-rap-8-13.pdf. [Last accessed on 25 Apr 2024].
39. Regulation on organic fertilizers and related products. (in Norwegian) Available from: https://lovdata.no/dokument/SF/forskrift/2003-07-04-951. [Last accessed on 25 Apr 2024].
Cite This Article
Export citation file: BibTeX | RIS
OAE Style
Gomiero A, Øysæd KB, Jaén-Gil A, Navrestad V, Skogerbø G. To what extent are wastewater treatment systems a gateway for microplastic particles in the aquatic and terrestrial environments?. Water Emerg Contam Nanoplastics 2024;3:13. http://dx.doi.org/10.20517/wecn.2023.63
AMA Style
Gomiero A, Øysæd KB, Jaén-Gil A, Navrestad V, Skogerbø G. To what extent are wastewater treatment systems a gateway for microplastic particles in the aquatic and terrestrial environments?. Water Emerging Contaminants & Nanoplastics. 2024; 3(2): 13. http://dx.doi.org/10.20517/wecn.2023.63
Chicago/Turabian Style
Gomiero, Alessio, Kjell Birger Øysæd, Adrián Jaén-Gil, Veslemøy Navrestad, Geir Skogerbø. 2024. "To what extent are wastewater treatment systems a gateway for microplastic particles in the aquatic and terrestrial environments?" Water Emerging Contaminants & Nanoplastics. 3, no.2: 13. http://dx.doi.org/10.20517/wecn.2023.63
ACS Style
Gomiero, A.; Øysæd KB.; Jaén-Gil A.; Navrestad V.; Skogerbø G. To what extent are wastewater treatment systems a gateway for microplastic particles in the aquatic and terrestrial environments?. Water. Emerg. Contam. Nanoplastics. 2024, 3, 13. http://dx.doi.org/10.20517/wecn.2023.63
About This Article
Special Issue
Copyright
Data & Comments
Data
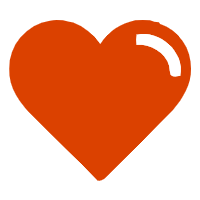

Comments
Comments must be written in English. Spam, offensive content, impersonation, and private information will not be permitted. If any comment is reported and identified as inappropriate content by OAE staff, the comment will be removed without notice. If you have any queries or need any help, please contact us at support@oaepublish.com.