Mechanism of metabolic surgery for the treatment of Type 2 Diabetes Mellitus (T2DM)
Abstract
After metabolic surgery, patients with type 2 diabetes (T2DM) typically experience a rapid improvement in glycemic control before any significant weight loss occurs. Furthermore, a significant proportion of patients are able to achieve long-term T2DM remission and improvement in
Keywords
INTRODUCTION
Almost 30 years ago, Pories et al. described their observations that morbidly obese patients rapidly improve their blood glucose control and experience remission of type 2 diabetes (T2DM) after Roux-en-Y gastric bypass (RYGB)[1]. Even more striking was that almost 90% maintained diabetes remission a decade later. Since then, many other investigators have demonstrated the benefits of metabolic surgery on T2DM[2-7]. Given the magnitude and rapidity by which glucose homeostasis is achieved and hyperglycemia is improved, the American Diabetes Association (ADA) recommends metabolic surgery to treat T2DM in severely obese patients and suggests its consideration even in the absence of severe obesity[8]. Therefore, there has been an increased emphasis placed on the underlying mechanisms by which metabolic surgery confers its benefits to T2DM.
Historically, metabolic surgery was thought to induce weight loss and thus, improvement in glycemic control by calorie restriction or malabsorption. For example, the improvement in T2DM control after laparoscopic adjustable gastric banding (LAGB) was mainly dependent on the proportion of weight loss[9,10]. While weight loss is a significant contributing factor, it is apparent that there are multiple important mechanisms that primarily regulate the glycemic benefit in T2DM patients after metabolic surgery. This can be observed in the difference in T2DM remission rates amongst different metabolic surgeries, such as LABG versus vertical sleeve gastrectomy (VSG) or RYGB[11,12].
Based on these observations, many mechanisms, in addition to calorie restriction and weight loss, have been proposed, so-called weight loss-independent mechanisms, to explain the improvement in T2DM after metabolic surgery versus diet-alone. These include changes in changes in bile acid (BA) metabolism, gastrointestinal (GI) hormone regulation, the intestinal microbiome, and physiologic mechanisms such as GI motility. While our understanding of metabolic surgery mechanisms on T2DM has increased significantly, it has also highlighted the complexity of the underlying factors involved. Consequently, the improvement in T2DM after metabolic surgery is more realistically an inter-play between all of these factors.
IMPACT OF CALORIE RESTRICTION AND WEIGHT LOSS
Within days of surgery, improvements in glucose homeostasis and control occur well before significant weight loss. As such, the early improvements in glucose control are unlikely attributable to total weight loss. This observation is one of the main reasons and part of the rationale for weight loss-independent mechanisms for glycemic improvement after metabolic surgery. Indeed, in studies of diabetic patients who had early matched weight loss after RYGB versus diet-alone, RYGB patients demonstrated greater oral glucose tolerance, as well as improvements in insulin resistance (IR) and sensitivity, compared to the diet-alone group[13,14].
While impressive, a few issues were identified with the design of these studies. There was a statistically significant difference in time to achieve equivalent weight loss between the groups, with diet-alone taking twice as long as RYGB. This would imply that the RYGB group had a significantly greater calorie restriction compared to the diet-alone group, which could potentially impact the results. Also, after RYGB, oral glucose reaches the intestine more quickly, resulting in earlier blood glucose and insulin peaks compared to diet-alone, which would make oral glucose tolerance testing (OGTT) difficult to compare.
In order to address these issues, Yoshino et al. devised an elegant study in which insulin sensitivity was tested before and after matched weight loss (~18%) in 22 obese T2DM patients who either underwent RYGB or diet-alone[15]. There was no significant difference in time to weight loss between the groups, and both groups had no differences in weight variability three weeks after weight loss, suggesting similar caloric intake/energy expenditure. Patients underwent intensive testing to assess tissue insulin sensitivity and insulin kinetics at the level of muscle, liver, and adipose tissue. Compared to before weight loss, both diet-alone and RYGB demonstrated decreased post-prandial plasma glucose, decreased endogenous glucose production, improved insulin sensitivity in the liver, skeletal muscle, and adipose tissue, and improved
Based on these results, it could be argued that calorie restriction, rather than weight loss, is the reason for early glycemic improvement. This argument is supported by several factors: (1) similar time to weight loss between both groups, suggesting similar caloric restriction; (2) both groups had a three-week post-weight loss period in which weight was maintained, indicating an equivalent energy balance; and (3) as previously stated, marked improvements in glucose control are seen after surgery and before significant weight loss. To this end, Jackness et al. sought to determine if the early glycemic effects of surgery were inherent to the procedure or were secondary to caloric restriction[16]. In this study, they compared patients who underwent RYGB with patients on a very low-calorie diet (VLCD) consisting of 500 kcal/day; the rationale being
While acute caloric restriction is important, it is well known that long-term weight loss leads to improvements in glycemic control in T2DM[17-20], highlighting the importance of weight loss in long-term glucose homeostasis. Knowing that obesity is a critical risk factor for T2DM development, research has demonstrated obesity-driven IR in white adipose tissue (WAT), liver, and skeletal muscle as a primary driver of T2DM. Furthermore, dysfunction of WAT in obese individuals is associated with disrupted metabolic homeostasis and increased risk for T2DM[21,22]. Visceral adipose tissue (VAT) has been increasingly implicated in the development of T2DM and metabolic syndrome (MetS)[21-23], further highlighting the importance of weight loss for long-term metabolic improvement.
Therefore, metabolic surgery can improve glycemic control in T2DM both acutely, through caloric restriction, and long-term through weight loss and a reduction in obesity-driven IR. However, there are factors uniquely induced by metabolic surgery that can lead to long-term durability of weight loss and improvements in glucose homeostasis. We will discuss this further in the following sections.
PHYSIOLOGIC ROLE OF GI HORMONES AND THE IMPACT OF SURGERY
Upon ingestion of food, a cascade of signals is triggered by the GI tract eliciting gut, neural, and hormonal responses. These various signals and responses are needed for digestion, but more importantly, they are critical in glucose and energy regulation. These signals are constantly incorporated along with peripheral signals related to energy stores and nutrient availability by the gut-brain axis, subsequently impacting nutrient utilization and influencing behavior. For example, leptin secreted from adipocytes and insulin serve as peripheral signals for long-term energy stores and can suppress appetite[22,24]. Conversely, ghrelin secreted in oxyntic glands of the fundus stimulates appetite and energy intake with increasing levels in the fasted state[25,26].
Many different peptide hormones are synthesized in enteroendocrine cells and secreted throughout the GI tract. The L cells, particularly in the distal intestine, produce glucagon-like peptide-1 (GLP-1) and peptide YY3-36 (PYY) and secrete them in response to nutrient ingestion[27]. Both GLP-1 and PYY exhibit appetite-suppressant effects[11,26], but GLP-1 is heavily implicated in glucose homeostasis. Oral glucose intake stimulates a greater insulin response in comparison to iso-glycemic parenteral glucose administration; this is known as the incretin effect and is largely mediated by GLP-1[11,27]. As such, GLP-1 receptor agonists (GLP-1 RAs) are a major pharmaceutical target in the management of T2DM.
In the obese state, there is a disruption of normal energy and glucose homeostatic signals, resulting in multiple pathophysiologic changes, including leptin and IR. Additionally, there is reduced circulating PYY and GLP-1 following nutrient intake and post-prandial suppression of ghrelin is reduced[11]. These changes function as a positive feed-back loop, bolstering appetite stimulation while impairing satiety, which perpetuates obesity, increasing IR and the risk of T2DM. Indeed, a decreased incretin effect is an early feature of T2DM[28].
While lifestyle intervention can lead to weight loss, long-term adherence is often poor, and many individuals experience weight regain[29]. This can be due to the compensatory changes in the body, including decreased energy expenditure[30], reduced circulating leptin, GLP-1, and PYY with increased levels of ghrelin[31]. This aligns with the evolutionary understanding that weight loss was typically associated with reduced survival. Interestingly, RYGB and VSG, in addition to inducing weight loss, are associated with reduced overall hunger[32].
To understand why the normal compensatory response of hunger was blunted in RYGB, Cummings et al. postulated that the surgery was impacting the circulating levels of ghrelin[33]. They compared ghrelin levels before and after weight loss in a diet-alone group versus the RYGB group and demonstrated that in the diet-alone group, there was a compensatory increase in ghrelin from baseline, whereas the surgery group, despite a greater weight loss, demonstrated a significantly reduced circulating ghrelin level. In VSG, the major source of ghrelin production, the gastric fundus, is excised and can explain the reduction in ghrelin levels[34].
It has now been widely demonstrated that following VSG, RYGB, and duodenal-jejunal bypass (DJB), there is marked elevation in GLP-1[35] and PYY[34] in comparison to restriction, whether caloric or from LABG. In RYGB, nutrients and bile bypass a significant portion of the intestinal tract and are delivered to the distal intestine, where a large portion of L cells reside, leading to the increase in GLP-1 and PYY. This has been demonstrated in numerous animal studies that have translated to humans[36-38]. Previously, the mechanism behind the elevation of GLP-1 and PYY after VSG was not completely understood, given the lack of GI diversion. However, in patients who have undergone VSG, multiple authors have demonstrated faster gastric emptying[39-41]. The mechanism behind this is thought to be potentially related to higher intraluminal pressures within the sleeve after surgery[42]. Interestingly, through the use of cine magnetic resonance imaging (MRI), there is increased small bowel motility independent of increased gastric emptying after VSG[39,43], which may explain how VSG increases post-prandial release of these GI peptides.
As previously discussed, despite elevations in GLP-1 following metabolic surgery, the initial improvement in glycemic control is most likely attributable to caloric restriction. However, as pointed out by Manning
With the abundance of studies investigating the relationship of GLP-1 and glucose control, it was also observed that GLP-1 RAs produced weight loss that was advantageous given that most T2DM patients are overweight or obese[51-53]. In a meta-analysis of 21 trials of obese or overweight patients with or without T2DM, those treated with GLP-1 RAs lost on average 2.9 kg compared to control; this weight loss was seen for both diabetics and non-diabetics[54]. While it is known that GLP-1 inhibits appetite[55,56], the complete mechanisms by which GLP-1 RAs mediate weight loss are not fully understood. In energy-deficient states, such as after metabolic surgery, higher levels of soluble leptin receptors are seen, which are thought to bind and sequester circulating leptin, thus inhibiting the appetite-reducing effect of hormones[57]. In a randomized controlled trial of 52 obese non-diabetic patients, Iepsen et al. showed that GLP-1 RA-treated patients had decreased appetite, greater weight loss, increased leptin, decreased soluble leptin receptor, and lower fasting glucose compared to control[58]. They also noted an increase in PYY meal response, suggesting that the appetite-inhibiting effect of GLP-1 RAs involves increased PYY levels.
These findings may also provide an understanding of the variation in weight loss after surgery. In one observational study following RYGB, patients were grouped into poor weight loss and good weight loss, with the former reporting less satiation and increased hunger along with lower PYY and GLP-1 and higher ghrelin in circulation compared to the latter group[59]. In support of gut hormones playing a role in weight loss following metabolic surgery, other studies have demonstrated that patients have increased appetite and weight gain with octreotide administration after RYGB[60] and blockade of both GLP-1 and PYY (but not either alone) counteracted the appetite-inhibition of RYGB, causing a ~20% increased food intake comparatively[61].
To summarize, following RYGB and VSG, there is an elevated post-prandial response involving GLP-1 and PYY with a decreased level of circulating ghrelin. GLP-1 RAs have been shown to induce weight loss and improve glycemic control; therefore, the exaggerated GLP-1 response following surgery may play a significant role in the long-term restoration of
GUT MICROBIOME AND METABOLIC SURGERY
The impact of the gut microbiome on health and disease is becoming increasingly apparent with alterations in the intestinal flora contributing to the development of several disorders[62]. Human studies have demonstrated that alterations in gut microbiome occur in metabolic diseases, including obesity and T2DM[63]. Furthermore, it is now understood that the gut microbiome has the ability to produce bioactive compounds that can elicit various responses in the host[64]. Considering this, there is significant interest in whether the gut microbiome contributes to the impact of metabolic surgery on glucose control.
Multiple studies have now demonstrated that metabolic surgery affects the composition and the activity of intestinal microbiota[65-68]. In one study comparing obese patients before and after RYGB, researchers observed increased diversity and altered composition of the gut microbiome in addition to weight loss and improved glycemic control after RYGB - a significant portion of these altered species was maintained long-term as well[69]. While weight loss from lifestyle modification is also associated with changes in the gut microbiota[70], metabolic surgery was able to augment the gut microbiome of obese individuals, making it more similar to the profile observed in lean individuals[65]. Most studies have demonstrated a relative reduction in Firmicutes and an increase of Bacteroidetes[67,69,71,72] with an increased Bacteroidetes to Firmicutes ratio reported after metabolic surgery in obese patients[65].
While metabolic surgery obviously influences the gut microbiome, it raises the question of whether these alterations contribute to the metabolic improvements following surgery. Multiple studies in mice have shown weight loss and decreased fat mass in germ-free mice after fecal transplants from mice and humans treated with metabolic surgery[73,74]. Similarly, Jahansouz et al. showed that after VSG in mice, exposure to antibiotics induced dysbiosis and resulted in significantly increased adiposity and impaired glucose homeostasis compared to control VSG mice[68].
Despite these findings in rodent studies, there is still a lack of understanding regarding the impact of the microbiome after metabolic surgery. Indeed, a recent systematic review conducted in 2019 revealed that while many alterations in the microbiome are described after surgery, there are few metabolic observations consistently reported across the studies[75]. While it seems that there is a profound effect on the microbiome following metabolic surgery, further research is needed to determine how this contributes to the metabolic effects after surgery. In the following section, we will discuss a potential mechanism by which the gut microbiome may impart a metabolic effect through its involvement in BA metabolism.
ROLE OF BILE ACIDS IN GLUCOSE AND LIPID METABOLISM IMPROVEMENT
BAs are organic acids synthesized from cholesterol in the liver and conjugated to taurine or glycine before excretion into bile. BAs are then released into the GI tract, where they function as surfactants in the digestion and absorption of lipids through micelle formation[73]. Following metabolic surgery in patients with obesity, it has been shown that the serum BA level is elevated. Although it is still controversial, there is an opinion that BA is one of the mechanisms for improving metabolism.
BAs are classified into primary BAs, which are synthesized in the liver, and secondary Bas, which are deconjugated by intestinal microbiota. In humans, the primary BAs are cholic acid (CA) and chenodeoxycholic acid (CDCA), while the secondary BAs include deoxycholic acid (DCA), lithocholic acid (LCA), and ursodeoxycholic acid (UDCA)[76]. More than 95% of BAs are actively reabsorbed in the GI tract, particularly in the distal ileum, through active transporters such as ileal BA transporter (IBAT) or apical sodium-dependent bile salt transporter (ASBT). These BAs then return to the liver via the portal vein. In the liver, BAs are taken up by active transporters, including sodium-taurocholate co-transporting polypeptide (NTCP) and organic anion transporting polypeptide (OATP)[77], into hepatocytes. They are subsequently excreted again into bile as conjugated BAs. This is referred to as the enterohepatic circulation of BAs.
While the synthesis of BAs is complex, it can be broken into two pathways. The classical pathway, which is normally responsible for at least 75% of BA production, involves the rate-limiting enzyme cholesterol 7
BAs have also been shown to regulate the expression of various genes via intracellular nuclear receptors and cell surface receptors[81-83]. Importantly, BAs serve as ligands for the farnesoid X receptor (FXR), a transcription factor that is involved in the initiation and regulation of various target gene expression[84]. FXR is expressed in multiple tissues, but its relevance is particularly notable in the liver and ileum[85], where activation can greatly affect BA transport. As previously described, primary BAs are synthesized through the enzymes CYP7A1 or CYP8B1; their expression is regulated by small heterodimeric partners (SHP), target proteins of FXR. Therefore, CYP7A1 and CYP8B1 are inhibited by SHP, which functions to prevent a rapid rise in BAs that is cytotoxic in vivo[81,86]. In addition, FXR activation promotes transcription of bile salt export pump (BSEP or ABCB11), multi-drug resistance protein 2 (MRP2), and multi-drug resistance protein 3 (MDR3). These are transporters that export BAs into the bile ducts from hepatocytes. Conversely, SHP suppresses the expression of NTCP and OATP, decreasing BA uptake from the portal vein[84].
In the small intestine, intestinal BA-binding protein (IBABP) is upregulated by FXR activation[84,87,88]. IBABP is involved in the transport of conjugated BAs, which are taken up by enterocytes via IBAT/ASBT, leading to the efficient handover of intracellular BAs to FXR[89,90]. In the presence of physiological concentrations of BAs, the activity and regulation of IBAT, IBABP, and FXR promote efficient BA uptake in the small intestine. When BAs accumulate excessively in cells, FXR activation increases organic solute transporter-α (OST-α) and -β (OST-β) expression on the basolateral side of enterocytes while suppressing IBAT expression on the apical side of enterocytes; the net action promotes BA traffic from the cells into the portal vein[84,87,88].
Given their responsibility for the formation of secondary BAs through modification of primary BAs, research has demonstrated that the gut microbiome plays a role in BA regulation and homeostasis as well. In terms of primary BA synthesis, it has been shown that the gut microbiome regulates multiple enzymes in both the classical and alternate synthesis pathways, including CYP7A1, CYP27A1, and CYP7B1. Furthermore, the microbiome is also involved in the regulation of taurine synthesis and one of the first enzymes necessary for BA conjugation[91]. In the gut, microbial deconjugation occurs through bile salt hydrolase (BSH), preventing the reuptake of primary BAs by ASBT. In the colon, these deconjugated BAs are then metabolized into secondary BAs that also have roles in cell signaling. However, this is a bidirectional relationship with BAs having the capability to alter the microbiome community. BAs can directly affect the microbiome pool by damaging bacterial membranes due to their detergent properties[92].
While BAs are involved in the regulation of their own transport and production through their interactions with FXR, they have additional functions as cell-signaling molecules. Specifically, BAs play a significant role in the regulation of glucose and lipid metabolism in the body. This is achieved through two pathways, which include the FXR-mediated pathway and their binding of transmembrane G-coupled protein receptor 5 (TGR5).
BAs are able to suppress liver gluconeogenesis through their binding of FXR. BA-bound FXR leads to the suppression of phosphoenolpyruvate carboxykinase (PEPCK) and glucose 6-phosphatase (G6Pase) expression in hepatocytes[93]. In ileal epithelial cells, the expression of fibroblast growth factor 19 (FGF19) increases due to FXR activation by BAs. FGF19 functions in an insulin-independent manner to promote hepatic protein synthesis and inhibit gluconeogenesis within the liver[94]. Additionally, FGF19 is involved in BA homeostasis through the inhibition of CYP7A1 and, thus, BA synthesis, demonstrating another way in which BAs regulate their synthesis.
In the liver, SHP, through the activation of FXR, influences lipid metabolism by its suppression of the transcription factor sterol response element binding protein-1c (SREBP-1c). SREBP-1c is involved in the regulation of fatty acid metabolism and suppresses triglyceride synthesis[95]. High levels of SREBP-1c have also been implicated in the development of hepatic IR through its inhibition of insulin receptor substrate-2 (IRS-2), an important feature of T2DM and MetS[96]. Therefore FXR, through downstream suppression of SREBP-1c, may improve IR[97]. Additionally, FXR activates peroxisome proliferator-activated receptor-α (PPAR-α) in hepatocytes, which is known to enhance fatty acid oxidation[98].
TGR5 is another target of BAs involved in host metabolism. TGR5 is a G-coupled protein receptor that is expressed in tissues such as the liver, GI tract, skeletal muscle, and brown adipocytes, as well as monocytes, macrophages, and Kupffer cells[99,100]. The main agonists for the receptor are the secondary BAs, LCA, and DCA, thus linking the gut microbiome to TGR5 activation. In brown adipose tissue, activation of TGR5 through BA binding promotes the conversion of thyroxine (T4) to triiodothyronine (T3) and induces the expression of uncoupling protein-1 (UCP-1), resulting in increased thermogenesis[101]. TGR5 activation in intestinal L cells has been shown to increase GLP-1 release[102,103] as well. In macrophages, activation of TGR5 suppresses lipopolysaccharide (LPS)-induced secretion of inflammatory cytokines, such as TNF-alpha, interleukin-1 (IL-1), IL-6, and IL-8[99].
Thus, BA signaling, either directly or through FXR/TGR5 and microbiome interactions, leads to (1) suppression of gluconeogenesis, suppression of lipogenesis, and improvement of IR through FXR pathway
Figure 1. Metabolism regulation through the FXR pathway. Red arrows indicated the elevated factors by FXR activation, while blue arrows indicated the decreased factors. Bile acid signaling suppresses gluconeogenesis and lipogenesis, stimulates fatty-acid oxidation, improves insulin resistance, and downregulates bile acid synthesis through the FXR pathway. ASBT: Apical sodium-dependent bile salt transporter; BSEP: bile salt export pump; CYP7A1: rate-limiting enzyme cholesterol 7 α-hydroxylase; FABP: fatty acid binding protein; FGFR: fibroblast growth factor receptor; FGF19: fibroblast growth factor 19; FXR: farnesoid X receptor; IBAT: ileal BA transporter; IRS-2: insulin receptor substrate-2; NTCP: sodium-taurocholate co-transporting polypeptide; OATP: organic anion transporting polypeptide; OST: organic solute transporter; PEPCK: phosphoenolpyruvate carboxykinase; PPAR-α: peroxisome proliferator-activated receptor-α; SHP: small heterodimeric partners; SREBP-1c: sterol response element binding protein-1c.
Figure 2. Metabolism regulation through the TGR5 pathway. Red arrows indicated the elevated factors by TGR5 activation, while blue arrows indicated the decreased factors. Bile acid signaling stimulates energy expenditure, suppresses inflammatory cytokine secretion, and increases GLP-1 secretion through the TGR5 pathway. cAMP: Fibroblast growth factor receptor; GLP-1: glucagon-like peptide-1; LPS: lipopolysaccharide; TGR5: transmembrane G-coupled protein receptor 5; UCP-1: uncoupling protein-1.
EFFECTS OF METABOLIC SURGERY ON BILE ACIDS
It is well known that serum BA levels are significantly increased in obese patients following metabolic surgery; for example, after RYGB, these levels are increased two-four years after surgery. These levels were correlated with the increased secretion of GLP-1 after surgery, along with a decrease in post-prandial blood glucose[73,104], demonstrating that BAs may be involved in these metabolic improvements. Similarly, FGF19 was seen to increase after RYGB and VSG, but no changes in BAs or FGF19 were observed in LABG or caloric restriction[105]. In patients with T2DM, circulating BAs and FGF19 are less than in normoglycemic individuals, while after RYGB, these levels are higher in those who have T2DM remission versus those who do not[106].
The mechanisms by which BAs are elevated following metabolic surgery are different depending on the procedure. In RYGB, the anatomic rearrangements lead to more direct delivery of bile to the ileum, resulting in increased BA reuptake[107] in a similar fashion to GLP-1. Interestingly, recent studies have demonstrated that longer biliopancreatic limb (BPL) length is more effective for weight loss and diabetes improvement[108,109]. In addition, several animal studies have similarly shown that a longer BPL in obese mice results in enhanced weight loss and improved glucose tolerance[110,111] and that these effects are strongly related to the increase in serum BA concentration after surgery[111,112]. Furthermore, in terms of the BA fraction after DJB, it was found that BAs with high affinity for FXR, such as CDCA and DCA, were increased[112].
In a rat model study in which DJB was performed, Ise et al. injected labeled BAs through the duodenal stump, and the number of labeled BAs excreted into the bile duct was measured to determine the rate of enterohepatic circulation[113]. Both rapid turnover of BAs and higher levels of peak BA concentration were observed in the long BPL model. They also revealed that the absorption of BAs in the small intestine was more efficient in the monomer form than in the micellar form. The monomer form is representative of BP-limb contents that are not mixed with food, whereas the micellar form represents intestinal contents mixed with lipids in the diet[114]. Therefore, the increase in serum BA concentration after gastric bypass may be attributed to the shortening of enterohepatic circulation as a result of the rapid reabsorption of digestate-free BAs in the long BPL due to prolonged contact with the intestinal epithelium in monomeric form [Figure 3].
Figure 3. Shortening of enterohepatic bile acid circulation after duodenal-jejunal bypass. Rapid reabsorption of bile acids in the long BPL due to long contact of un-micellized bile acids to the intestinal epithelium seemed essential. AL: Alimentary limb; BA: bile acid; BPL: biliopancreatic limb; CC: common channel.
Elevations in BA levels have been demonstrated in VSG in a nonhuman primate (NHP) study[96] as well as in patients[115-117], though more modest than in RYGB or DJB. As discussed earlier, there is increased intragastric pressure leading to faster gastric emptying as well as increased gut motility following VSG. This reduces the mixing of BA with food before reaching the distal intestine and may explain the rise in serum BA levels. In mice, decreased ghrelin was shown to enhance the IBAT expression in the intestine[118]. It is known that VSG leads to a sustained reduction in ghrelin levels due to removal of the fundus[26,34] and thus may further explain the elevated serum BA levels after surgery.
Understanding the impact of BAs on the regulation of glucose and lipid metabolism in the body provides mechanistic reasons behind the improvements following metabolic surgery aside from weight loss and caloric restriction. Elevations in BAs after surgery can further suppress gluconeogenesis and improve IR through the FXR and FGF19 pathways while increasing energy expenditure and GLP-1 secretion via TGR5. Interestingly, VSG has been shown to induce the differentiation of intestinal stem cells into enteroendocrine cells in mice. While unclear if this translates to humans, this effect could be mediated through FXR-BA signaling and may result in an increase in GLP-1-positive cells. Many of these studies have been conducted in models such as rodents, and it should be noted that there is room for debate as to whether it is appropriate to directly apply them to the mechanism of metabolic improvement after metabolic surgery in humans.
IMPACT OF VISCERAL ADIPOSE TISSUE AND INFLAMMATION ON DIABETES
It was briefly discussed how weight loss following metabolic surgery could improve glycemic control through a reduction in WAT. Again, WAT and, specifically, VAT have been implicated in the development of IR and T2DM. However, the mechanism by which this occurs is complex and is a major focus in diabetes and MetS research. Furthermore, understanding the impact of metabolic surgery on these mechanisms has also garnered significant attention.
It is now understood that a key driver of the peripheral and hepatic IR in T2DM is due to adipose tissue inflammation, specifically local inflammation in VAT[22,96]. Macrophages have been implicated in the control and development of VAT inflammation and have classically been described phenotypically as M2 (anti-inflammatory) versus M1 (pro-inflammatory)[119,120]. These M2-like macrophages work in concern with resident regulatory T-cells (Tregs) in VAT to control inflammation and IR[119]. In mouse models of obesity, decreased M2-like macrophages and resident Tregs are associated with VAT inflammation and IR, while in lean mice, VAT is enriched with these cell populations[119,121]. The observations that several clusters of macrophage subpopulations exist and that there may be associations between the presence of lipid-associated macrophages and metabolic disease open the door to further study of the role of bariatric surgery in this context.
Interestingly, BAs have been shown to control immune cell phenotypes, including macrophage polarization and Treg differentiation[122]. Furthermore, as has been discussed, obesity and T2DM alter the normal gut microbial communities. This suggests that the bidirectional interaction between the gut microbiome and BAs influences host immunity and inflammation, providing another mechanism by which metabolic surgery might impact glucose hemostasis.
To expand upon this theory, Nugent et al. reported the results of VSG versus sham surgery in an NHP model and monitored various outcomes for one year, including weight loss, glucose metabolism parameters, gut hormones, microbiome, metabolites, and immune cell phenotypes[96]. They demonstrated durable weight loss with a reduction in adiposity, increased post-prandial secretion, microbiome changes, and elevations in BA levels mimicking outcomes in patients. Of great interest was the impact of VSG on VAT inflammation. Early changes within one month demonstrated a shift in VAT immunocytes towards a regulatory phenotype, with VSG inducing expansion of regulatory macrophages and Tregs. These immune changes were evident up to a year later, suggesting that VSG can modulate the obesogenic memory of VAT.
VAGAL NERVE PHYSIOLOGY AND IMPACT OF SURGERY
The vagus nerve is known to influence upper GI function and metabolism[123]. Afferent vagal nerve fibers are sensitive to the mechanical stretch of the stomach after ingestion of food. Furthermore, gut hormones, including ghrelin, GLP-1, and PYY, have been suggested to interact with vagal nerve receptors[124]. Studies investigating the impact of intermittent vagal nerve blockade on weight loss have shown some promise, but more work is necessary before drawing conclusions[125,126]. It has been shown that after RYGB and VSG, gastric branches of the vagus nerve are cut, causing damage to efferent and afferent fibers[124]. The significance of this and whether or not it is related to the metabolic effects of surgery require further investigation.
CONCLUSIONS
While impressive improvements in glycemic control and remission of T2DM have been observed following metabolic surgery for decades, the underlying mechanisms remained unclear for nearly as long. Previously, the anti-diabetes effect of metabolic surgery was once believed to be purely related to weight loss and calorie restriction; however, it is now abundantly clear that a multitude of complex factors underlies this effect. Studies have demonstrated that the rapid improvement in glycemic control in the immediate post-operative period is due to the caloric restriction imparted by surgery. Long-term improvement in
Following metabolic surgery, there exists a complex cross-talk between gut hormones, BAs, gut microbiome, the immune system, and adipose tissue, all of which contribute to the efficacy of the surgery and its improvements in glycemic control. While many mechanisms have been identified, more investigation is necessary to completely understand how all these systems interact to improve patient health.
DECLARATIONS
Authors’ contributionsMade substantial contributions to the conception and design of the study and performed data analysis and interpretation: Leishman DJ, Naitoh T, Ikkramuddin S
Performed data acquisition and provided administrative, technical, and material support: Leishman DJ, Naitoh T, Ikkramuddin S
Availability of data and materialsNot Applicable.
Financial support and sponsorshipNone.
Conflicts of interestAll authors declared that there are no conflicts of interest.
Ethical approval and consent to participateNot applicable.
Consent for publicationNot applicable.
Copyright© The Author(s) 2023.
REFERENCES
1. Pories WJ, Swanson MS, MacDonald KG, et al. Who would have thought it? An operation proves to be the most effective therapy for adult-onset diabetes mellitus. Ann Surg 1995;222:339-50; discussion 350-2.
2. Brethauer SA, Aminian A, Romero-Talamás H, et al. Can diabetes be surgically cured? Long-term metabolic effects of bariatric surgery in obese patients with type 2 diabetes mellitus. Ann Surg 2013;258:628-36; discussion 636-7.
3. Cohen RV, Pinheiro JC, Schiavon CA, Salles JE, Wajchenberg BL, Cummings DE. Effects of gastric bypass surgery in patients with type 2 diabetes and only mild obesity. Diabetes Care 2012;35:1420-8.
4. DeMaria EJ, Sugerman HJ, Kellum JM, Meador JG, Wolfe LG. Results of 281 consecutive total laparoscopic Roux-en-Y gastric bypasses to treat morbid obesity. Ann Surg 2002;235:640-5; discussion 645-7.
5. Gill RS, Birch DW, Shi X, Sharma AM, Karmali S. Sleeve gastrectomy and type 2 diabetes mellitus: a systematic review. Surg Obes Relat Dis 2010;6:707-13.
6. Schauer PR, Burguera B, Ikramuddin S, et al. Effect of laparoscopic Roux-en Y gastric bypass on type 2 diabetes mellitus. Ann Surg 2003;238:467-84; discussion 84-5.
7. Ikramuddin S, Korner J, Lee WJ, et al. Roux-en-Y gastric bypass vs intensive medical management for the control of type 2 diabetes, hypertension, and hyperlipidemia: the diabetes surgery study randomized clinical trial. JAMA 2013;309:2240-9.
8. ElSayed NA, Aleppo G, Aroda VR, et al. 8. Obesity and weight management for the prevention and treatment of type 2 diabetes: standards of care in diabetes-2023. Diabetes Care 2023;46:S128-39.
9. Dixon JB, O’Brien PE, Playfair J, et al. Adjustable gastric banding and conventional therapy for type 2 diabetes: a randomized controlled trial. JAMA 2008;299:316-23.
10. Pok EH, Lee WJ. Gastrointestinal metabolic surgery for the treatment of type 2 diabetes mellitus. World J Gastroenterol 2014;20:14315-28.
11. Batterham RL, Cummings DE. Mechanisms of diabetes improvement following bariatric/metabolic surgery. Diabetes Care 2016;39:893-901.
12. Inabnet WB 3rd, Winegar DA, Sherif B, Sarr MG. Early outcomes of bariatric surgery in patients with metabolic syndrome: an analysis of the bariatric outcomes longitudinal database. J Am Coll Surg 2012;214:550-6; discussion 556-7.
13. Laferrère B, Teixeira J, McGinty J, et al. Effect of weight loss by gastric bypass surgery versus hypocaloric diet on glucose and incretin levels in patients with type 2 diabetes. J Clin Endocrinol Metab 2008;93:2479-85.
14. Plum L, Ahmed L, Febres G, et al. Comparison of glucostatic parameters after hypocaloric diet or bariatric surgery and equivalent weight loss. Obesity 2011;19:2149-57.
15. Yoshino M, Kayser BD, Yoshino J, et al. Effects of diet versus gastric bypass on metabolic function in diabetes. N Engl J Med 2020;383:721-32.
16. Jackness C, Karmally W, Febres G, et al. Very low-calorie diet mimics the early beneficial effect of Roux-en-Y gastric bypass on insulin sensitivity and
17. Wing RR, Koeske R, Epstein LH, Nowalk MP, Gooding W, Becker D. Long-term effects of modest weight loss in type II diabetic patients. Arch Intern Med 1987;147:1749-53.
18. Bosello O, Armellini F, Zamboni M, Fitchet M. The benefits of modest weight loss in type II diabetes. Int J Obes Relat Metab Disord 1997;21 Suppl 1:S10-3. Available from: https://pubmed.ncbi.nlm.nih.gov/9130035/. [Last accessed on 19 Jul 2023]
19. Sjöström CD, Peltonen M, Wedel H, Sjöström L. Differentiated long-term effects of intentional weight loss on diabetes and hypertension. Hypertension 2000;36:20-5.
20. Wing RR, Lang W, Wadden TA, et al. Look AHEAD Research Group. Benefits of modest weight loss in improving cardiovascular risk factors in overweight and obese individuals with type 2 diabetes. Diabetes Care 2011;34:1481-6.
21. Kusminski CM, Bickel PE, Scherer PE. Targeting adipose tissue in the treatment of obesity-associated diabetes. Nat Rev Drug Discov 2016;15:639-60.
22. Longo M, Zatterale F, Naderi J, et al. Adipose tissue dysfunction as determinant of obesity-associated metabolic complications. Int J Mol Sci 2019;20:2358.
23. Fox CS, Massaro JM, Hoffmann U, et al. Abdominal visceral and subcutaneous adipose tissue compartments: association with metabolic risk factors in the Framingham heart study. Circulation 2007;116:39-48.
24. Makaronidis JM, Batterham RL. Obesity, body weight regulation and the brain: insights from fMRI. Br J Radiol 2018;91:20170910.
26. Pucci A, Batterham RL. Mechanisms underlying the weight loss effects of RYGB and SG: similar, yet different. J Endocrinol Invest 2019;42:117-28.
28. Ahrén B. Incretin dysfunction in type 2 diabetes: clinical impact and future perspectives. Diabetes Metab 2013;39:195-201.
29. Wing RR, Bolin P, Brancati FL, et al. Look AHEAD Research Group. Cardiovascular effects of intensive lifestyle intervention in type 2 diabetes. N Engl J Med 2013;369:145-54.
30. Leibel RL, Hirsch J. Diminished energy requirements in reduced-obese patients. Metabolism 1984;33:164-70.
31. Sumithran P, Prendergast LA, Delbridge E, et al. Long-term persistence of hormonal adaptations to weight loss. N Engl J Med 2011;365:1597-604.
32. Manning S, Pucci A, Batterham RL. Roux-en-Y gastric bypass: effects on feeding behavior and underlying mechanisms. J Clin Invest 2015;125:939-48.
33. Cummings DE, Weigle DS, Frayo RS, et al. Plasma ghrelin levels after diet-induced weight loss or gastric bypass surgery. N Engl J Med 2002;346:1623-30.
34. Yousseif A, Emmanuel J, Karra E, et al. Differential effects of laparoscopic sleeve gastrectomy and laparoscopic gastric bypass on appetite, circulating acyl-ghrelin, peptide YY3-36 and active GLP-1 levels in non-diabetic humans. Obes Surg 2014;24:241-52.
35. Manning S, Pucci A, Batterham RL. GLP-1: a mediator of the beneficial metabolic effects of bariatric surgery? Physiology 2015;30:50-62.
36. Dirksen C, Damgaard M, Bojsen-Møller KN, et al. Fast pouch emptying, delayed small intestinal transit, and exaggerated gut hormone responses after Roux-en-Y gastric bypass. Neurogastroenterol Motil 2013;25:346-e255.
37. Johannessen H, Kodama Y, Zhao CM, et al. Eating behavior and glucagon-like peptide-1-producing cells in interposed ileum and pancreatic islets in rats subjected to ileal interposition associated with sleeve gastrectomy. Obes Surg 2013;23:39-49.
38. Ramzy AR, Nausheen S, Chelikani PK. Ileal transposition surgery produces ileal length-dependent changes in food intake, body weight, gut hormones and glucose metabolism in rats. Int J Obes 2014;38:379-87.
39. Melissas J, Leventi A, Klinaki I, et al. Alterations of global gastrointestinal motility after sleeve gastrectomy: a prospective study. Ann Surg 2013;258:976-82.
40. Sioka E, Tzovaras G, Perivoliotis K, et al. Impact of laparoscopic sleeve gastrectomy on gastrointestinal motility. Gastroenterol Res Pract 2018;2018:4135813.
41. Vives M, Molina A, Danús M, et al. Analysis of gastric physiology after laparoscopic sleeve gastrectomy (LSG) with or without antral preservation in relation to metabolic response: a randomised study. Obes Surg 2017;27:2836-44.
42. Yehoshua RT, Eidelman LA, Stein M, et al. Laparoscopic sleeve gastrectomy - volume and pressure assessment. Obes Surg 2008;18:1083-8.
43. Trung VN, Yamamoto H, Furukawa A, et al. Enhanced intestinal motility during oral glucose tolerance test after laparoscopic sleeve gastrectomy: preliminary results using cine magnetic resonance imaging. PLoS One 2013;8:e65739.
44. Dutia R, Brakoniecki K, Bunker P, et al. Response to comment on Dutia et al. Limited recovery of β-cell function after gastric bypass despite clinical diabetes remission. Diabetes 2014;63:1214-23.
45. Anderwald CH, Tura A, Promintzer-Schifferl M, et al. Alterations in gastrointestinal, endocrine, and metabolic processes after bariatric Roux-en-Y gastric bypass surgery. Diabetes Care 2012;35:2580-7.
46. Näslund E, Grybäck P, Hellström PM, et al. Gastrointestinal hormones and gastric emptying 20 years after jejunoileal bypass for massive obesity. Int J Obes 1997;21:387-92.
47. Jiménez A, Casamitjana R, Viaplana-Masclans J, Lacy A, Vidal J. GLP-1 action and glucose tolerance in subjects with remission of type 2 diabetes after gastric bypass surgery. Diabetes Care 2013;36:2062-9.
48. Jørgensen NB, Dirksen C, Bojsen-Møller KN, et al. Exaggerated glucagon-like peptide 1 response is important for improved β-cell function and glucose tolerance after Roux-en-Y gastric bypass in patients with type 2 diabetes. Diabetes 2013;62:3044-52.
49. Salehi M, Prigeon RL, D’Alessio DA. Gastric bypass surgery enhances glucagon-like peptide 1-stimulated postprandial insulin secretion in humans. Diabetes 2011;60:2308-14.
50. Chambers AP, Jessen L, Ryan KK, et al. Weight-independent changes in blood glucose homeostasis after gastric bypass or vertical sleeve gastrectomy in rats. Gastroenterology 2011;141:950-8.
51. Gerich J. Pathogenesis and management of postprandial hyperglycemia: role of incretin-based therapies. Int J Gen Med 2013;6:877-95.
52. Inzucchi SE, Bergenstal RM, Buse JB, et al. Management of hyperglycaemia in type 2 diabetes: a patient-centered approach. Position statement of the American diabetes association (ADA) and the European association for the study of diabetes (EASD). Diabetologia 2012;55:1577-96.
53. Ryan D, Acosta A. GLP-1 receptor agonists: nonglycemic clinical effects in weight loss and beyond. Obesity 2015;23:1119-29.
54. Vilsbøll T, Christensen M, Junker AE, Knop FK, Gluud LL. Effects of glucagon-like peptide-1 receptor agonists on weight loss: systematic review and meta-analyses of randomised controlled trials. BMJ 2012;344:d7771.
55. Astrup A, Rössner S, Van Gaal L, et al. NN8022-1807 Study Group. Effects of liraglutide in the treatment of obesity: a randomised, double-blind, placebo-controlled study. Lancet 2009;374:1606-16.
56. Flint A, Raben A, Astrup A, Holst JJ. Glucagon-like peptide 1 promotes satiety and suppresses energy intake in humans. J Clin Invest 1998;101:515-20.
57. Kratzsch J, Lammert A, Bottner A, et al. Circulating soluble leptin receptor and free leptin index during childhood, puberty, and adolescence. J Clin Endocrinol Metab 2002;87:4587-94.
58. Iepsen EW, Lundgren J, Dirksen C, et al. Treatment with a GLP-1 receptor agonist diminishes the decrease in free plasma leptin during maintenance of weight loss. Int J Obes 2015;39:834-41.
59. Dirksen C, Jørgensen NB, Bojsen-Møller KN, et al. Gut hormones, early dumping and resting energy expenditure in patients with good and poor weight loss response after Roux-en-Y gastric bypass. Int J Obes 2013;37:1452-9.
60. le Roux CW, Welbourn R, Werling M, et al. Gut hormones as mediators of appetite and weight loss after Roux-en-Y gastric bypass. Ann Surg 2007;246:780-5.
61. Svane MS, Jørgensen NB, Bojsen-Møller KN, et al. Peptide YY and glucagon-like peptide-1 contribute to decreased food intake after Roux-en-Y gastric bypass surgery. Int J Obes 2016;40:1699-706.
62. Hartstra AV, Bouter KE, Bäckhed F, Nieuwdorp M. Insights into the role of the microbiome in obesity and type 2 diabetes. Diabetes Care 2015;38:159-65.
63. Arora T, Bäckhed F. The gut microbiota and metabolic disease: current understanding and future perspectives. J Intern Med 2016;280:339-49.
64. Holmes E, Li JV, Marchesi JR, Nicholson JK. Gut microbiota composition and activity in relation to host metabolic phenotype and disease risk. Cell Metab 2012;16:559-64.
65. Damms-Machado A, Mitra S, Schollenberger AE, et al. Effects of surgical and dietary weight loss therapy for obesity on gut microbiota composition and nutrient absorption. Biomed Res Int 2015;2015:806248.
66. Furet JP, Kong LC, Tap J, et al. Differential adaptation of human gut microbiota to bariatric surgery-induced weight loss: links with metabolic and low-grade inflammation markers. Diabetes 2010;59:3049-57.
67. Jahansouz C, Staley C, Bernlohr DA, Sadowsky MJ, Khoruts A, Ikramuddin S. Sleeve gastrectomy drives persistent shifts in the gut microbiome. Surg Obes Relat Dis 2017;13:916-24.
68. Jahansouz C, Staley C, Kizy S, et al. Antibiotic-induced disruption of intestinal microbiota contributes to failure of vertical sleeve gastrectomy. Ann Surg 2019;269:1092-100.
69. Palleja A, Kashani A, Allin KH, et al. Roux-en-Y gastric bypass surgery of morbidly obese patients induces swift and persistent changes of the individual gut microbiota. Genome Med 2016;8:67.
70. Nadal I, Santacruz A, Marcos A, et al. Shifts in clostridia, bacteroides and immunoglobulin-coating fecal bacteria associated with weight loss in obese adolescents. Int J Obes 2009;33:758-67.
71. Graessler J, Qin Y, Zhong H, et al. Metagenomic sequencing of the human gut microbiome before and after bariatric surgery in obese patients with type 2 diabetes: correlation with inflammatory and metabolic parameters. Pharmacogenomics J 2013;13:514-22.
72. Kong LC, Tap J, Aron-Wisnewsky J, et al. Gut microbiota after gastric bypass in human obesity: increased richness and associations of bacterial genera with adipose tissue genes. Am J Clin Nutr 2013;98:16-24.
73. Liu H, Hu C, Zhang X, Jia W. Role of gut microbiota, bile acids and their cross-talk in the effects of bariatric surgery on obesity and type 2 diabetes. J Diabetes Investig 2018;9:13-20.
74. Tremaroli V, Karlsson F, Werling M, et al. Roux-en-Y gastric bypass and vertical banded gastroplasty induce long-term changes on the human gut microbiome contributing to fat mass regulation. Cell Metab 2015;22:228-38.
75. Davies NK, O’Sullivan JM, Plank LD, Murphy R. Altered gut microbiome after bariatric surgery and its association with metabolic benefits: a systematic review. Surg Obes Relat Dis 2019;15:656-65.
76. Russell DW. The enzymes, regulation, and genetics of bile acid synthesis. Annu Rev Biochem 2003;72:137-74.
77. Slijepcevic D, van de Graaf SF. Bile acid uptake transporters as targets for therapy. Dig Dis 2017;35:251-8.
79. Thomas C, Pellicciari R, Pruzanski M, Auwerx J, Schoonjans K. Targeting bile-acid signalling for metabolic diseases. Nat Rev Drug Discov 2008;7:678-93.
80. Li-Hawkins J, Gåfvels M, Olin M, et al. Cholic acid mediates negative feedback regulation of bile acid synthesis in mice. J Clin Invest 2002;110:1191-200.
81. Lu TT, Makishima M, Repa JJ, et al. Molecular basis for feedback regulation of bile acid synthesis by nuclear receptors. Mol Cell 2000;6:507-15.
82. Makishima M, Okamoto AY, Repa JJ, et al. Identification of a nuclear receptor for bile acids. Science 1999;284:1362-5.
83. Parks DJ, Blanchard SG, Bledsoe RK, et al. Bile acids: natural ligands for an orphan nuclear receptor. Science 1999;284:1365-8.
84. Teodoro JS, Rolo AP, Palmeira CM. Hepatic FXR: key regulator of whole-body energy metabolism. Trends Endocrinol Metab 2011;22:458-66.
85. Lefebvre P, Cariou B, Lien F, Kuipers F, Staels B. Role of bile acids and bile acid receptors in metabolic regulation. Physiol Rev 2009;89:147-91.
86. Wahlström A, Sayin SI, Marschall HU, Bäckhed F. Intestinal crosstalk between bile acids and microbiota and its impact on host metabolism. Cell Metab 2016;24:41-50.
87. Lee FY, Lee H, Hubbert ML, Edwards PA, Zhang Y. FXR, a multipurpose nuclear receptor. Trends Biochem Sci 2006;31:572-80.
88. Urizar NL, Dowhan DH, Moore DD. The farnesoid X-activated receptor mediates bile acid activation of phospholipid transfer protein gene expression. J Biol Chem 2000;275:39313-7.
89. Trauner M, Boyer JL. Bile salt transporters: molecular characterization, function, and regulation. Physiol Rev 2003;83:633-71.
90. Campana G, Pasini P, Roda A, Spampinato S. Regulation of ileal bile acid-binding protein expression in Caco-2 cells by ursodeoxycholic acid: role of the farnesoid X receptor. Biochem Pharmacol 2005;69:1755-63.
91. Sayin SI, Wahlström A, Felin J, et al. Gut microbiota regulates bile acid metabolism by reducing the levels of tauro-beta-muricholic acid, a naturally occurring FXR antagonist. Cell Metab 2013;17:225-35.
92. Inagaki T, Moschetta A, Lee YK, et al. Regulation of antibacterial defense in the small intestine by the nuclear bile acid receptor. Proc Natl Acad Sci U S A 2006;103:3920-5.
93. Zhang Y, Lee FY, Barrera G, et al. Activation of the nuclear receptor FXR improves hyperglycemia and hyperlipidemia in diabetic mice. Proc Natl Acad Sci U S A 2006;103:1006-11.
94. Kir S, Beddow SA, Samuel VT, et al. FGF19 as a postprandial, insulin-independent activator of hepatic protein and glycogen synthesis. Science 2011;331:1621-4.
95. Watanabe M, Houten SM, Wang L, et al. Bile acids lower triglyceride levels via a pathway involving FXR, SHP, and SREBP-1c. J Clin Invest 2004;113:1408-18.
96. Nugent JL, Singh A, Wirth KM, et al. A nonhuman primate model of vertical sleeve gastrectomy facilitates mechanistic and translational research in human obesity. iScience 2021;24:103421.
97. Ide T, Shimano H, Yahagi N, et al. SREBPs suppress IRS-2-mediated insulin signalling in the liver. Nat Cell Biol 2004;6:351-7.
98. Preidis GA, Kim KH, Moore DD. Nutrient-sensing nuclear receptors PPARα and FXR control liver energy balance. J Clin Invest 2017;127:1193-201.
99. Kawamata Y, Fujii R, Hosoya M, et al. A G protein-coupled receptor responsive to bile acids. J Biol Chem 2003;278:9435-40.
100. Maruyama T, Tanaka K, Suzuki J, et al. Targeted disruption of G protein-coupled bile acid receptor 1 (Gpbar1/M-Bar) in mice. J Endocrinol 2006;191:197-205.
101. Watanabe M, Houten SM, Mataki C, et al. Bile acids induce energy expenditure by promoting intracellular thyroid hormone activation. Nature 2006;439:484-9.
102. Thomas C, Gioiello A, Noriega L, et al. TGR5-mediated bile acid sensing controls glucose homeostasis. Cell Metab 2009;10:167-77.
103. Katsuma S, Hirasawa A, Tsujimoto G. Bile acids promote glucagon-like peptide-1 secretion through TGR5 in a murine enteroendocrine cell line STC-1. Biochem Biophys Res Commun 2005;329:386-90.
104. Patti ME, Houten SM, Bianco AC, et al. Serum bile acids are higher in humans with prior gastric bypass: potential contribution to improved glucose and lipid metabolism. Obesity 2009;17:1671-7.
105. Sachdev S, Wang Q, Billington C, et al. FGF 19 and bile acids increase following Roux-en-Y gastric bypass but not after medical management in patients with type 2 diabetes. Obes Surg 2016;26:957-65.
106. Gerhard GS, Styer AM, Wood GC, et al. A role for fibroblast growth factor 19 and bile acids in diabetes remission after Roux-en-Y gastric bypass. Diabetes Care 2013;36:1859-64.
107. Kohli R, Kirby M, Setchell KD, et al. Intestinal adaptation after ileal interposition surgery increases bile acid recycling and protects against obesity-related comorbidities. Am J Physiol Gastrointest Liver Physiol 2010;299:G652-60.
108. Eskandaros MS, Abbass A. Standard biliopancreatic limb (50 cm) Roux-en-Y gastric bypass versus long biliopancreatic limb
109. Zerrweck C, Herrera A, Sepúlveda EM, Rodríguez FM, Guilbert L. Long versus short biliopancreatic limb in Roux-en-Y gastric bypass: short-term results of a randomized clinical trial. Surg Obes Relat Dis 2021;17:1425-30.
110. Schneider R, Kraljević M, Peterli R, et al. Roux-en-Y gastric bypass with a long versus a short biliopancreatic limb improves weight loss and glycemic control in obese mice. Surg Obes Relat Dis 2022;18:1286-97.
111. Miyachi T, Nagao M, Shibata C, et al. Biliopancreatic limb plays an important role in metabolic improvement after duodenal-jejunal bypass in a rat model of diabetes. Surgery 2016;159:1360-71.
112. Tsuchiya T, Naitoh T, Nagao M, et al. Increased bile acid signals after duodenal-jejunal bypass improve non-alcoholic steatohepatitis (NASH) in a rodent model of diet-induced NASH. Obes Surg 2018;28:1643-52.
113. Ise I, Tanaka N, Imoto H, et al. Changes in enterohepatic circulation after duodenal-jejunal bypass and reabsorption of bile acids in the bilio-pancreatic limb. Obes Surg 2019;29:1901-10.
114. Ueno T, Tanaka N, Imoto H, et al. Mechanism of bile acid reabsorption in the biliopancreatic limb after duodenal-jejunal bypass in rats. Obes Surg 2020;30:2528-37.
115. Steinert RE, Peterli R, Keller S, et al. Bile acids and gut peptide secretion after bariatric surgery: a 1-year prospective randomized pilot trial. Obesity 2013;21:E660-8.
116. Seeley RJ, Chambers AP, Sandoval DA. The role of gut adaptation in the potent effects of multiple bariatric surgeries on obesity and diabetes. Cell Metab 2015;21:369-78.
117. Kindel TL, Krause C, Helm MC, et al. Increased glycine-amidated hyocholic acid correlates to improved early weight loss after sleeve gastrectomy. Surg Endosc 2018;32:805-12.
118. Kang K, Schmahl J, Lee JM, et al. Mouse ghrelin-O-acyltransferase (GOAT) plays a critical role in bile acid reabsorption. FASEB J 2012;26:259-71.
120. Shapouri-Moghaddam A, Mohammadian S, Vazini H, et al. Macrophage plasticity, polarization, and function in health and disease. J Cell Physiol 2018;233:6425-40.
121. Becker M, Levings MK, Daniel C. Adipose-tissue regulatory T cells: critical players in adipose-immune crosstalk. Eur J Immunol 2017;47:1867-74.
122. Hang S, Paik D, Yao L, et al. Bile acid metabolites control TH17 and Treg cell differentiation. Nature 2019;576:143-8.
123. Berthoud HR. Vagal and hormonal gut-brain communication: from satiation to satisfaction. Neurogastroenterol Motil 2008;20 Suppl 1:64-72.
124. Stefanidis A, Oldfield BJ. Neuroendocrine mechanisms underlying bariatric surgery: insights from human studies and animal models. J Neuroendocrinol 2017;29:e12534.
125. Ikramuddin S, Blackstone RP, Brancatisano A, et al. Effect of reversible intermittent intra-abdominal vagal nerve blockade on morbid obesity: the ReCharge randomized clinical trial. JAMA 2014;312:915-22.
Cite This Article
Export citation file: BibTeX | RIS
OAE Style
Leishman DJ, Ikramuddin S, Naitoh T. Mechanism of metabolic surgery for the treatment of Type 2 Diabetes Mellitus (T2DM). Mini-invasive Surg 2023;7:27. http://dx.doi.org/10.20517/2574-1225.2023.29
AMA Style
Leishman DJ, Ikramuddin S, Naitoh T. Mechanism of metabolic surgery for the treatment of Type 2 Diabetes Mellitus (T2DM). Mini-invasive Surgery. 2023; 7: 27. http://dx.doi.org/10.20517/2574-1225.2023.29
Chicago/Turabian Style
Leishman, David J, Sayeed Ikramuddin, Takeshi Naitoh. 2023. "Mechanism of metabolic surgery for the treatment of Type 2 Diabetes Mellitus (T2DM)" Mini-invasive Surgery. 7: 27. http://dx.doi.org/10.20517/2574-1225.2023.29
ACS Style
Leishman, DJ.; Ikramuddin S.; Naitoh T. Mechanism of metabolic surgery for the treatment of Type 2 Diabetes Mellitus (T2DM). Mini-invasive. Surg. 2023, 7, 27. http://dx.doi.org/10.20517/2574-1225.2023.29
About This Article
Special Issue
Copyright
Data & Comments
Data
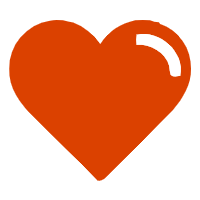

Comments
Comments must be written in English. Spam, offensive content, impersonation, and private information will not be permitted. If any comment is reported and identified as inappropriate content by OAE staff, the comment will be removed without notice. If you have any queries or need any help, please contact us at support@oaepublish.com.